by Joel Pedro, Centre for Ice and Climate
My recent paper in Geophysical Research Letters (co-led with Torge Martin from Kiel) was in part inspired by discussions at the ice2ice supported ‘MIS3/Southern Ocean and Bipolar Seesaw Workshop’ held in Bergen in January 2015. Check out the paper for the details; read on for a little story telling.
Dansgaard-Oeschger events, in their most iconic form, are saw-toothed 5–16°C temperature variations in Greenland ice cores1,2. Now, more than 30 years after these abrupt climate changes were first seen in the Greenland ice3, we have learned that D-O variability echoes through the entire climate system: in the colour of river sediments in Venezuela4, in water isotope variations in speleothems from China5, in glacial advance and retreat in Patagonia6 and in Antarctic ice cores7, where we see gradual warming of several degrees while Greenland is in the cold (stadial) sate and then gradual cooling periods while Greenland is in the warm (interstadial) state (Fig 1).
Pin the tail on the Donkey
So where does the process start? Palaeoclimatologists have been playing pin the tail on the donkey with DO events for years (I include myself here). This is how you play (thanks Wikepedia11):
“One at a time, each child is blindfolded and handed a paper “tail” with a push pin or thumbtack poked through it. The blindfolded child is then spun around until he or she is disoriented. The child gropes around and tries to pin the tail on the donkey. The game, a group activity, is generally not competitive; “winning” is only of marginal importance.”
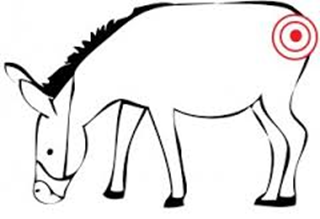
And so we mark our territory. The Laurentide ice sheet bristles with tacks12, as does the North Atlantic13. Clusters of tacks pepper the tropical pacific14, the Nordic seas15 and few decorate Antarctica16 and the Agulhas17. A la mode, the latest are stuck into volcanoes18. Some miss the board entirely19.
So where do we stick the tack with this paper? Well, I’m from Australia and co-author Sune Rasmussen had a sabbatical there and fell in love with the outback and Aussie pies; so naturally we stuck it in the Southern Ocean. Then the reviewers spun us around again and we became less decisive… but first things first, why care about the Southern Ocean during D-Os?
Global sea level20, atmospheric CO2 (ref 21) and (in breaking results from Jeff Severinghuas’ group at SCRIPPS) global ocean heat content22, all vary in phase with the Antarctic temperature trend during D-Os. The fact that these curves look like the Antarctic record does not prove that the southern high latitudes dominate the processes involved in CO2, sea level and total ocean heat content. But the dominance of the Antarctic signal in these rather fundamental measures of the climate state certainly does demand we pay attention to what is going on in the south; if it walks like a duck…
The prevailing explanation for the phasing of the Greenland and Antarctic temperature trends during D-Os is the bipolar ocean seesaw hypothesis23. According to this view D-Os are due to changes in northward ocean heat transport. Increased heat transport is said to cause abrupt warming in the North Atlantic and Greenland (with some help from sea-ice feedbacks) at the same time as gradually depleting the heat reservoir of the Southern Ocean and cooling Antarctica24; vice-versa, weakened northward ocean heat transport is said to cause abrupt cooling in Greenland and gradual warming in the Southern Ocean and Antarctica. This concept works nicely in box models and in many intermediate complexity models25. But it runs into trouble when confronted with a more realistic depiction of the ocean, in which the steeply outcropping isopycnals of the Antarctic Circumpolar Current (ACC) present a barrier to heat transport between the South Atlantic and Southern Ocean. The ACC may not be an insurmountable barrier, but anomalies certainly doesn’t go across nice and easy26.
For additional motivation to look to the south consider that the Southern Ocean currently takes up 75% of anthropogenic heat and 40% of anthropogenic CO2 (ref. 27). Whether the Southern Ocean will continue to provide this service for us is one of the biggest uncertainties in future temperature and sea level rise projections. Understanding what happens in the south during D-O’ seems like a meaningful challenge for the modellers and dynamicists who are trying to predict what the Southern Ocean may do in the future.
Polynyas: windows to the deep ocean
So getting to the point, our GRL paper describes a process that could drive past Antarctic warming events and conceivably even CO2 variations actively from the Southern Ocean itself.
Our idea was inspired in part by microwave satellite data from the 1970’s that shows three consecutive years in which there is a huge ice free area (250,000 km2) in the normally ice-packed Weddell Sea28. The Germans got a ship into this ‘open ocean polynya’ and found that the mixed layer extended to the sea floor29. The sea ice was being kept at bay by massive convective heat loss. The heat supply to sustain the deep convection was supplied by the intermediate depths of the Southern Ocean. The polynya shut down after 1976 and has not reappeared since. However some oceanographers suspect the sort of deep ocean convection that was responsible for the Weddell Polynya may have been more common in the past.
According to Arnold L. Gordon, leader of the original German cruise to the polynya in the 70’s, ocean deep convection may have been the only way for the deep Southern Ocean to vent its heat during the glacial period—because glacial ice cover would have shut down margin processes30. So now we have a potential mechanism to drive Antarctic warming that doesn’t depend on propagating temperature anomalies across the ACC. This was especially attractive to our ‘ACC is a barrier to heat transport’ enthusiast co-author Markus Jochum.
Meanwhile, Torge Martin who I met at U. Washington, while I was a postdoc there, showed me some free-running simulations with the Kiel Climate Model that display some very interesting behaviour in the Southern Ocean. In a 2,000-year free-running simulation, heat accumulates at Southern Ocean intermediate depths and then is released to the atmosphere via deep convection—in the Weddell Sea! The convection lasts until the ocean heat reservoir is depleted, which takes several centuries. Convection then shuts down while the intermediate depths re-accumulate heat. Eventually the threshold of thermal instability is crossed, warm deep water is entrained into the mixed layer, and convection gets off and running again.
Southern Ocean internal variability and Antarctic Warming
In our paper we show that heat loss from the convective zone in the Weddell Sea ultimately causes warming of up to 2°C on the Antarctic continent. Four factors are important (in almost equal measure) in driving the Antarctic warming: ocean to atmosphere heat flux from the convective zone; sea-ice loss and albedo feedback; southward migration of the ACC; and increased heat and moisture transport to Antarctica. The southward migration of the ACC, which takes around 50 years, was a surprise. We put this migration down to heat (i.e. buoyancy) loss from the convective zone dragging the outcropping isopycnals southward. A southward-shifted ACC can also be viewed as a southward shift in the sub-tropical front, which in turn means warmer sea surface temperatures in the mid-latitudes. In response to the mid-latitude warming the atmosphere fluxes more heat toward Antarctica. Fascinating, isn’t it! The behaviour is summed up in our abstract like this:
- Simulations with a free-running coupled climate model show that heat release associated with Southern Ocean deep convection variability can drive centennial-scale Antarctic temperature variations of up to 2.0°C. The mechanism involves three steps: Preconditioning: heat accumulates at depth in the Southern Ocean; Convection onset: wind and/or sea-ice changes tip the buoyantly unstable system into the convective state; and Antarctic warming: fast sea-ice—albedo feedbacks (on annual-decadal time scales) and slow Southern Ocean frontal and sea surface temperature adjustments to convective heat release (on multidecadal-century time scales) drive an increase in atmospheric heat and moisture transport toward Antarctica. We discuss the potential of this mechanism to help drive and amplify climate variability as observed in Antarctic ice-core records.
The two-stage response of the southern high latitudes to open ocean deep convection is illustrated in the schematic below (Fig 3.)
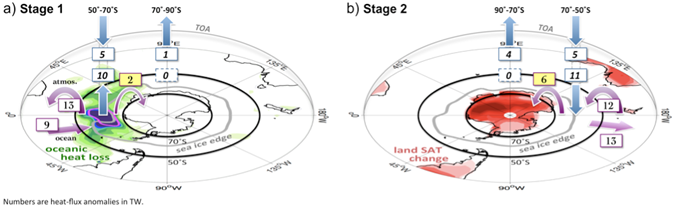
Ok, so where’s the link to the north?
As our reviewers pointed out, the mechanism above could be an interesting form of internal climate variability without necessarily having anything to do with D-Os! It’s a good point and I can’t rule out that they are right (yet). So on to some speculation.
Accounting for the time relationship between the Antarctic and Greenland temperature records across the D-O events would call for a process to push the southern high latitude system toward the deep-convecting warming mode while Greenland is in cold stadial conditions (and vice versa). During Greenland stadials we have good evidence that the Inter Tropical Convergence Zone and the Southern Ocean surface wind field are both shifted south5,31. Southward shifted winds would indeed be expected to push the Southern Ocean system toward the convective state by enhancing the Ekman-driven upwelling of intermediate-depth waters, enhancing the advection of sea ice northward away from the convective zone, and deepening the mixed layer32. The situation would be reversed in the case of Greenland interstadials in which there is evidence for a northward shift in the ITCZ and Southern Ocean surface wind field.
Hence we present a chain of coupled ocean and atmosphere processes that would help to bridge the oceanic barrier formed by the ACC. Some things we want to examine next are whether the deep convection events could also release CO2 to the atmosphere and whether the recharge of the heat reservoir that sustains the deep convection events may be linked to the strength or depth of the Atlantic Overturning.
So what about the donkey? Well, at this stage I’m still hanging on to my tack and increasingly of a mind that trying to define where the process starts may not be the most constructive way to tackle the D-O problem. A better approach may be to try to identify the necessary processes and to reject the processes that conflict with the data (e.g. as appears the case with meltwater forcing33) or that conflict with the physics (as may be the case with the bipolar ocean seesaws reliance on propagating anomalies across the ACC). Watch this space.
Comments and complaints to: jpedro@nbi.ku.dk
References
- NGRIP members (2004), High-resolution record of Northern Hemisphere climate extending into the Last Interglacial period, Nature 431, 147.
- Kindler, P. et al. (2014), Temperature reconstruction from 10 to 120 kyr b2k from the NGRIP ice core, Clim. Past 10, 887–902.
- Dansgaard, W. et al. (1982), New Greenland Deep Ice Core, Science 218 (4579), 1273.
- Peterson, L.C. et al. (2000), Rapid Changes in the Hydrologic Cycle of the Tropical Atlantic During the Last Glacial, Science 290, 1947.
- Wang, Y. J. et al. (2001), A High-Resolution Absolute-Dated Late Pleistocene Monsoon Record from Hulu Cave, China, Science 294, 2345.
- Moreno, P.I. et al. (2009), Renewed glacial activity during the Antarctic Cold Reversal and persistence of cold conditions until 11.5 ka in SW Patagonia, Geology 37, 375.
- EPICA Community Members (2006), One-to-one coupling of glacial climate variability in Greenland and Antarctica, Nature 444, 195.
- Parrenin, F. et al. (2013), Synchronous change of atmospheric CO2 and Antarctic temperature during the last deglacial warming, Science 339(6123), 1060.
- Rasmussen, S. O. et al. (2014), A stratigraphic framework for abrupt climatic changes during the last glacial period based on three synchronized Greenland ice-core records: Refining and extending the INTIMATE event stratigraphy. Quat. Sci. Rev. 106, 14.
- WAIS Divide Project Members (2015), Precise interpolar phasing of abrupt climate change during the last ice age, Nature 520, 661
- https://en.wikipedia.org/wiki/Pin_the_tail_on_the_donkey
- MacAyeal, D.R. (1993), Binge/purge oscillations of the Laurentide Ice Sheet as a cause of the North Atlantic’s Heinrich events, Paleoceanography 8(6), 775.
- Broecker, W.S., D.M. Peteet, and D. Rind (1985), Does the ocean-atmosphere system have more than one stable mode of operation? Nature 315, 21.
- Kleppin, H. et al. (2015), Stochastic atmospheric forcing as a cause of Greenland climate transitions, J. Clim. 28, 7741.
- Dokken, T. M. et al. (2013), Interactions between ocean and sea ice intrinsic to the Nordic Seas, Paleoceanography, 28, 491.
- Weaver, A. J. et al. (2003), Meltwater Pulse 1A from Antarctica as a Trigger of the Bølling-Allerød Warm Interval, Science 299, 1709.
- Marino, G. et al. (2013), Agulhas salt-leakage oscillations during abrupt climate changes of the Late Pleistocene, Paleoceanography 28, 599.
- Baldini, J.U., R.J. Brown & J.N. McElwaine (2015), Was millennial scale climate change during the Last Glacial triggered by explosive volcanism? Nature Scientific Reports, 5:17442.
- Braun, H. et al. (2005), Possible solar origin of the 1470-year glacial climate cycle demonstrated in a coupled model, Nature 438, 208.
- Siddall, M., E.J. Rohling, W.G. Thompson & C. Waelbroeck (2008), Marine isotope stage 3 sea level fluctuations: Data synthesis and new outlook, Rev. Geophys. 46.
- Bereiter, B. et al. (2012), Mode change of millennial CO2 variability during the last glacial cycle associated with a bipolar marine carbon seesaw, Proc. Nat. Acad. of Sci.109(25), 9755.
- Bereiter, B. et al. (2016), Mean ocean temperature change over the last transition based on atmospheric changes in heavy noble mixing ratios, International Partnerships in Ice Core Science, Hobart, March 2016.change during the last ice age, Nature 520, 661.
- Crowley, T.J. (1992), North Atlantic deep water cools the Southern Hemisphere, Paleoceanography 7, 489.
- Stocker, T. F. & S. J. Johnsen (2003), A minimum thermodynamic model for the bipolar seesaw, Paleoceanography 18(4), 1087.
- Schmittner, A., O.A. Saenko & A.J. Weaver (2003), Coupling of the hemispheres in observations and simulations of glacial climate change, Quat. Sci. Rev. 22, 659.
- Ferrari, R., & M.J. Nikurashin (2010), Suppression of eddy diffusivity across jets in the Southern Ocean, J. Phys. Oceanogr. 40, 1501.
- Frölicher, T.L. et al. (2015), Dominance of the Southern Ocean in anthropogenic carbon and heat uptake in CMIP5 models, J. Clim., 28, 862–886, doi:10.1175/JCLI-D-14-00117.1.
- Carsey, F. D. (1980), Microwave observation of the Weddell polynya, Mon. Weather Rev. 108, 2032.
- Gordon, A.L. (1982), Weddell deep water variability, J. Mar. Res. 40, 199.
- Gordon, A.L. (2014), Oceanography: Southern Ocean polynya, Nat. Clim. Change 4(4), 249.
- Montade, V. et al. (2015), Teleconnection between the Intertropical Convergence Zone and southern westerly winds throughout the last deglaciation, Geology 43(8), 735.
- Cheon, W.G., Y.-G. Park, J.R. Toggweiler & S.-K. Lee (2014), The relationship of Weddell polynya and open-ocean deep convection to the southern hemisphere westerlies, J. Phys. Oceanogr., 44, 694.
- Barker, S.C. et al. (2015), Icebergs not the trigger for North Atlantic cold events, Nature 520(7547), 333.